Earliest Signs of Parkinson's Disease with D. James Surmeier, PhD
Northwestern Medicine scientists have discovered one of the earliest signs of Parkinson's disease, proving that damaged neuronal mitochondria alone can cause symptoms of the disease, according to a study published in Nature. Senior author D. James Surmeier, PhD, chair of the Feinberg department of Neuroscience, who has over 30 years of experience in the field, explains the importance of these findings for future Parkinson's research and therapeutics.
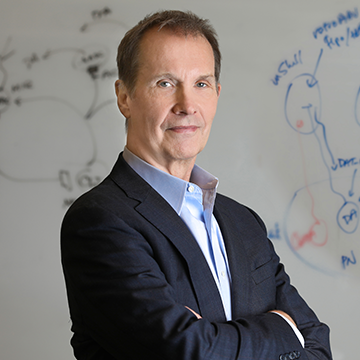
"We have the potential with this model to identify biomarkers and behavioral markers that might help us identify early-stage Parkinson's patients."
- Chair of the Department of Neuroscience
- Nathan Smith Davis Professor of Neuroscience
- Member of Northwestern University Clinical and Translational Sciences Institute (NUCATS)
Episode Notes
mitochondria in dopamine neurons is enough to cause the disease. Using a new disease model in mice, the team replicated the slow progression of the disease in humans.
opening the door to new therapies for late-stage Parkinson’s disease patients.
Topics covered:
- One of the molecular markers of the disease is the decline of mitochondrial function in dopamine neurons in the basal ganglia, though whether this decline is a cause or a consequence of the disease was unknown. This study shows the decline is not just a "tombstone" but a cause.
- The team developed a new disease model in mice that mimicked the staged progression of Parkinson's disease in humans and which related regional loss of dopamine with behavior.
- Standard of care for late-stage Parkinson's patients is the drug levodopa, which Surmeier says is quite effective for motor symptoms. As the disease progresses and more dopaminergic neurons are lost, however, the drug becomes less effective. In the study, the team used gene therapy to target the area in the brain where these neurons reside, the substantia nigra, and substantially boosted benefits of the drug.
- Surmeier recently received a $9 million grant from the Aligning Science Across Parkinson’s initiative through the Michael J. Fox Foundation for further study of the dysfunction of basal ganglia circuits and their connection to motor and sleep symptoms.
Additional Reading:
-
Disruption of mitochondrial complex I induces progressive parkinsonism in Nature
-
Determinants of seeding and spreading of α-synuclein pathology in the brain in Science Advances
- Dopamine metabolism by a monoamine oxidase mitochondrial shuttle activates the electron transport chain in Nature Neuroscience
Subscribe to Feinberg School of Medicine podcasts here:
iTunes
Spotify
Google Play Music
Recorded on Oct. 29, 2021
Amanda Dee: Northwestern Medicine scientists have discovered one of the earliest signs of Parkinson's disease, proving that damaged neuronal mitochondria alone can cause symptoms of the disease. According to a study published in Nature, senior author D. James Surmeier, chair of the Feinberg department of Neuroscience, who has over 30 years of experience in the field, explains the importance of these findings for future Parkinson's research and therapeutics. Welcome to the show.
D. James Surmeier: Thank you for having me.
Amanda Dee: So the overall goal of your lab is to understand how basal ganglia function affects behavior and how it's involved in neurodegenerative diseases, like Parkinson's. What is the role of basal ganglia and what drew you to study them?
D. James Surmeier: Basal ganglia are a very ancient part of the brain. It's 500 million years old. You find the basal ganglia and animals as low on the evolutionary tree as lampreys. And the overall goal of the basal ganglia is to help us do the right thing in the right circumstance to promote our survival. So typically people will talk about the basal ganglia as helping us move in a way that achieves our goals and avoids punishments. It also is key to habits. One of the things that it does is to help us, again, automate behavior. So it sort of takes a little bit of the burden off of the cerebral cortex, so we can walk and chew gum at the same time, things like that. But again, it helps automate behavior in important ways that are based upon experience. I got interested in the basal ganglia for two reasons.
One is I had an uncle who died of Parkinson's disease and that really puzzled me at the time. I really wasn't a very serious neuroscientist. I was a mathematician at the time, but it stuck with me. And then after my first post-doctoral fellowship, I got the opportunity to study with one of the world's experts in the basal ganglia, and he enticed me to come to his lab to study what dopamine does. And dopamine again is the key neurotransmitter in the basal ganglia that is lost in Parkinson's disease. But dopamine at the time was very puzzling because it didn't work in the way transmitters normally work. It didn't simply produce excitation or inhibition the way glutamate or GABA does, but rather it worked through what are called G protein coupled receptors acting at the surface of neurons to modulate the way in which cells responded to those classical transmitters. So it was a very mysterious chemical substance in the brain at the time. And over the course of the last 30 years, we've learned a lot about how it and other neuromodulators work. The brain is full of neuromodulators, like dopamine. Dopamine just turns out to be particularly important to a wide variety of disease states like Parkinson's disease.
Amanda Dee: So Parkinson's disease affects millions of people worldwide with symptoms that can progress from tremors to loss of motor function. If we were to zoom in to the molecular level on what your lab focuses, what does disease progression look like?
D. James Surmeier: Well, Parkinson's disease is a multifactorial disease. It involves many parts of the brain, we know that now. But the core motor symptoms that you described, the slowness of movement, the rigidity, are caused by the slow progressive degeneration of dopaminergic neurons, which reside in the mesencephalon and innovate a key part of the basal ganglia called the striatum as well as other parts of the basal ganglia – and that's actually a very important part of this story. What begins to happen in the early stages of the disease is, from our study of humans, we know that the axons, that is the part of a neuron that communicates with other cells, it begins to become dysfunctional. It stops releasing dopamine at the same level as it used to. And so, again, the communication between dopaminergic neurons and the rest of the basal ganglia becomes impaired.
With progression of the disease, you see the emergence of the motor symptoms that slow slowness of movement, but at the, at the molecular level, we really have not had a very good handle on what causes each stage of the disease. So again, we know from study of humans, that axons go first and then cell bodies go first, but we don't know how that relates to the symptoms of the disease. This is one of the big advances of the model that we've been able to develop. In terms of other molecular events that happen, we know that mitochondrial dysfunction is critical to the beginnings of Parkinson's disease. The thing that we have not had a very clear picture of, though, is whether mitochondrial dysfunction – now mitochondria are the power plants inside neurons – we think that the brain depends critically upon mitochondria. When you breathe, you're providing oxygen to the mitochondria to produce ATP, which is the sort of energy molecule inside of neurons that allows neurons to do all the variety of things that it does to generate action potentials, to release transmitter, to grow and to maintain itself.
But we know that mitochondrial function begins to decline. What we've not known is whether or not that's cause or a consequence of the disease. One of the things that I think has become clear from our work, and that of many other groups, is that in Parkinson's disease, there's an interaction between natural function of certain parts of the brain in particular, the dopaminergic neurons, and what we normally think of as disease mechanisms, genetic mutations, environmental toxins, things like that. These neurons have a phenotype that puts their mitochondria under stress all of the time. This is a natural occurrence in these cells. So our idea was that somehow that natural design, which led to basal mitochondrial stress, interacted with factors that began to diminish mitochondrial function to eventually push these cells over a bioenergetic cliff , and they just would stop working. One of those, of course, is the number one risk factor for Parkinson's disease, which is age.
The average age of diagnosis with PD is about 60. We knew that whatever was driving the disease took a long time to manifest itself. This is even true in most genetic forms of Parkinson's disease. And so we know with age that the power plants inside neurons, particularly in dopaminergic neurons, which have this high basal metabolic activity, that the mitochondria begin to become dysfunctional with age. So the hypothesis was that there was an interaction between that basal stress and some other factor that was pushing the disease forward. The prevailing view, although it's not proven, is that they have a basal mitochondrial oxidant stress because they have massive axonal arbors that control wide swaths of the brain, in particular the striatum is a very large part of the brain. And so individual neurons control large segments of that. And as a consequence, they have very large axons, which have lots of transmitter release sites, lots of sites that have to be supplied with building blocks and also sites where once proteins and lipids become damaged, those have to be degraded.
So the way I think about it, these cells are like a small town in Iowa trying to support the food and degradative needs of the city of Chicago. And it really puts a stress on that small little town in Iowa to do that. Whereas most neurons, most cities in Iowa, have to support other small towns in Iowa, or Indiana, right? So these neurons are different in that they're having to support this massive axonal arbor that spans a large region of the brain. It's sort of interesting that many of the other neurons that are vulnerable in Parkinson's disease have a very similar phenotype, that is, they also are responsible for controlling large areas of the brain. They all release neuromodulators, so they're all involved. They're almost like supervising the control over large areas of the brain and that sort of CEO phenotype creates a basal stress on them that creates wear and tear over time and we think ultimately leads to their demise. There's lots of natural cell loss with aging, more cell loss with healthy aging of this cell population than most other neurons in the brain. And it's true of many of the other vulnerable cell types in Parkinson's disease.
Amanda Dee: And your team published a study in Nature on this wear and tear that leads to the disruption of the mitochondrial complex I. What did you set out to address in the study and what did you find?
D. James Surmeier: There are many people who feel as though mitochondrial dysfunction is a driver of the disease, but quite frankly, the scientific literature on this point was controversial and inconclusive. There are many people who had attempted to manipulate mitochondrial function inside not just dopaminergic neurons but other neurons and not found a Parkinsonian phenotype when they did this in dopaminergic neurons. And so there's a sizeable population of people in the scientific community that basically think that the mitochondrial damage that you see in humans is really just a marker of the disease – it was like a tombstone of the disease, but wasn't a real cause. And so this is the thing that we set out to test. I mean, we continued to believe that mitochondrial dysfunction was in fact a driver, that supplying the energy necessary to maintain the massive structural features of dopaminergic neurons was something that could easily lead to their demise.
The other thing that is important to note here is that there are many ways in which you can manipulate mitochondrial function and cause neuronal death. So in the past, people had used toxins. The trouble with that model is that it happens way too fast. It doesn't mimic the natural course of Parkinson's disease, which again, takes decades and decades to manifest itself. So what we set out to do was to use a different strategy to test the mitochondrial hypothesis, because again, that model that toxin model had been used consistently to test therapeutics that were then taken to the clinic and they failed, time and time again. So we knew that that model, even though it implicated mitochondria, was not translating to the human disease. And so there was the argument that mitochondria are not really involved, that that's just a red herring, it's just a trick that you're playing on the brain that really doesn't reflect what's going on or the disease. So we used a genetic strategy. We deleted a gene that codes for a key subunit of mitochondrial complex I. Now mitochondrial complex I is one of five subunits in the electron transport chain of mitochondria, which are essential to the generation of ATP and mitochondrial complex I is the first, obviously, but it also is the one that manifests the greatest damage in human brains, both with aging and in Parkinson's disease. So what we did is we knocked out the gene for the subunit. And mitochondrial proteins are very long-lived, so they turn over very slowly. So when we knocked out the gene, the proteins that that gene codes for stayed around for a long period of time. Now this, actually, turned out to be critical to the outcome of the experiment because it gave cells the opportunity to adapt.
This is what is happening in Parkinson's. When we did that, there was a miraculous reprogramming of the transcriptome, or the genes that dopaminergic neurons were expressing. They very slowly switched off genes associated with mitochondrial production of ATP and turned up the genes associated with another way in which cells can generate ATP. This is through a process called glycolysis. Now glycolysis is not as efficient, but it nevertheless generates ATP. And if you up-regulate it enough, you can generate enough ATP to sustain many cell functions. However, the thing that happened, even though the cells survived, their axons became dysfunctional. That is, that massive axonal arbor that releases dopamine throughout the basal ganglia was not capable of being sustained by glycolysis alone. This mimicked what we see in humans, that is the axon is the most vulnerable part of the cell is the first thing that goes.
So it's consistent with the idea that mitochondrial dysfunction is a driver of pathogenesis and Parkinson's because we're mimicking something that happens in the human disease. Slowly though, with time, the somatodendritic region of the cell began to be dysfunctional. And although it became dysfunctional, what it was doing was it was sort of just stopping. It was kind of going into retirement. The cells stop doing their jobs and down-regulated proteins associated with transmitter release in particular, but didn't die. And this is, again, another key feature of Parkinson's disease where dopaminergic neurons and the mesencephalon of Parkinson's disease patients have stopped producing dopamine, stopped doing their jobs, but they're hanging out. Many of those neurons continue to be there even in late-stage Parkinson's disease patients, maybe as many as 30 percent of the dopaminergic neurons or neurons that were once dopaminergic are still there.
So there's another feature of the disease that we've mimicked in this model. The other key thing about this model because of this progressive human-like staging, that is the axons becoming dysfunctional first and then slowly the somatodendritic region becoming dysfunctional, it allowed us to relate regional loss of dopamine with behavior. Something that we would love to been able to do in humans, but we don't have longitudinal studies of humans because we only know you're going to get Parkinson's disease once you've manifested late-stage symptoms. We don't have any marker for prodromal or very early-stage Parkinson's disease, but we had it in this mouse because we had this nice, slow, progressive dysfunction that we could map with histological techniques and molecular techniques and physiological techniques. And it reveals something astonishing to us. And this really is the thing that has a wide variety of translational implications.
So for 30 years, for as long as I've been in the field, we've thought that when dopamine release in the striatum failed, that's when you became Parkinsonian, that's when you would have slowness of moment, that's when you would have rigidity, that's when you would have all those other kind of core motor features of the disease. But in this mouse, when we saw, very early on, loss of striatal dopamine release, the mice were not Parkinsonian at all. They had learning deficits. So I told you at the outset: one of the things that the basal ganglia does is to help us learn goal-directed activity, right? To do things that lead to reward and avoid things that lead to punishment. So we took those mice and food-deprived them and put them in a Y maze and put food over on the left. Now, for most mice, that have intact and functional basal ganglia, that's a one-trial learning event. You'd take them out, and the next day you'd put them back into the maze and they'd go [rushing sound effect], straight to where the food was, or the cheese was. These mice could not remember where the food was, so that goal-directed behavior was lost. Now early, if we gave them a little bit more dopamine, we could restore that behavior. Later, we couldn't restore that behavior by giving levodopa because the axons that go to the striatum had, we think, begun to degenerate by that point. The other thing that the animals had a little bit of difficulty doing was sort of fine, sensory guided tasks, like removing a sticker from their paw. They didn't like that, they would pull at the sticker and pull it off. But if you put them out on the floor and let them run around, you couldn't tell them from normal mice.
Now, as the disease progressed and the somatodendritic region began to become dysfunctional, that's when the Parkinsonian symptoms themselves. Now, dopaminergic neurons are really interesting. They're different from most neurons in the brain in that most neurons in the brain release neurotransmitter just from their axon. That's the way they communicate with other cells. But dopaminergic neurons also release neurotransmitter from their dendrites and from their soma. So they communicate with other neurons in the brain, not just where their axon goes, but also where their cell body sits. And the basal ganglia is designed in such a way that the cell bodies of these dopaminergic neurons sit right at the place where all of the information from the basal ganglia flows out to the rest of the brain. The striatum doesn't communicate with other parts of the brain. Its information processing flows out of that spot. It's a little bit like a funnel. So the striatum is large, its information is passed down to the output of the basal ganglia, and at that mouth is where the dopaminergic neurons sit. So the bottom line is it looked like to us that dopamine release from the somatodendritic region was critical to the emergence of the Parkinsonian symptoms. That has huge translational implications for a wide variety of reasons that we can get into.
Amanda Dee: And in addition to the translational or potential clinical possibilities from this study, how do you think this will affect the framework overall of Parkinson's research? This is a huge finding. Do you think that this is going to change the way that scientists approach their work?
D. James Surmeier: Well, one of the things it does is it makes clear that dopamine is acting in other parts of the basal ganglia than just the striatum. I mean, it also helps clarify the role of mitochondria and how they might fit into a broader pathogenic scheme for Parkinson's disease, as well as other neurodegenerative diseases. I don't want to leave the audience with the idea, though, that the only way to cause Parkinson's disease is through mitochondrial dysfunction. This is an existence statement. This is a demonstration that mitochondrial dysfunction is sufficient. It's not a demonstration that all forms of Parkinsonism are caused through this mechanism. Now that said, the other prevailing view is that misfolded forms of a protein that is enriched in those axons and those transmitter release sites, which because of the massive axonal arbor is expressed at very high levels in dopaminergic neurons and other at-risk neurons, that misfolded forms of that protein poison cells and ultimately kill them.
And I think that there's a lot of translational work being done right now to try and control the spread of misfolded forms of alpha-synuclein. And I think that there's a lot of merit to that hypothesis. That said, one of the things that we've begun to do is to look at the effects of misfolded forms of alpha-synuclein and mitochondrial function. And in fact, one of the things that it does is it exacerbates the stress on mitochondria and could hasten their demise. So it could very well be the case that the two things are working in concert with one another and both promoting pathogenesis and Parkinson's disease through complementary mechanisms in terms of disease modification. And there are no means by which we can slow the progression of Parkinson's disease at this point. It helps us understand what are the sequence of events that lead to not just mitochondrial dysfunction, but early axonal loss and then subsequent loss of the somatodendritic region of dopaminergic neurons.
It also helps us understand why those other neurons that are vulnerable in Parkinson's disease that lead to deficits in attention or deficits in autonomic function or deficits in cognitive function, why they may be vulnerable and leads to, points us toward strategies that might help to slow their decline with aging. It also gives us a strategy for identifying patients that might be particularly amenable to disease-modifying therapies. And why do I say that? Again, as I said at the beginning, we have no way of identifying people who are going to develop Parkinson's disease in five or 10 years. If we could do that, we might be able to start interventions with them that would slow the progression of the disease. And rather than transitioning to a clinical phase of Parkinson's disease in five years, it might be 15 years. And since the average age of diagnosis is 60, now you're beginning to push the limits of longevity. And so we have the potential with this model to be able to identify biomarkers and behavioral markers that might help us identify early stage patients, but there are other translational implications of this as well. One that's in the paper is very exciting and I'm hoping actually makes it to the clinic very soon. And that's related to, again, this regional depletion and the emergence of Parkinson's symptoms. One of the things that happens when you get diagnosed with Parkinson's disease, the standard of care is to give the precursor of dopamine – it's a substance called levodopa – to you orally. You can take it in tablet format. It's very effective in alleviating the symptoms of Parkinson's disease or the motor symptoms, anyway, because it boosts the production of dopamine by all of the dopaminergic neurons that remain. But it doesn't alter disease course.
So as you lose more and more dopaminergic neurons, it becomes less and less effective. So patients have to take more and more levodopa to the same level of symptomatic benefit. Typically within five to 10 years of a diagnosis with PD, the amount of levodopa that you're having to take is so high. You begin to have unwanted side effects. Ironically, the side effect is unwanted movement, dyskinesias. So at that point, patients have relatively few options. One of the options that they have right now is deep brain stimulation, or you can implant electrodes into a part of the brain called the subthalamic nucleus, which is a part downstream of the striatum, part of the basal ganglia, to regularize activity there. One of the things that happens as the disease progresses, as you get more and more aberrant activity in the basal ganglia, it starts poisoning other motor control circuits.
So this stimulation calms that down, it diminishes that aberrant activity, but it's still neurosurgery. I mean it's a very effective treatment and Northwestern actually is one of the centers of the world doing deep brain stimulation, we have some outstanding neurosurgeons here, but it would be better to have an alternative. One of the therapeutic strategies that has been attempted is to use a gene therapy to boost the production of an enzyme that converts levodopa to dopamine in the brain. With this gene therapy, you can get cells that wouldn't normally convert levodopa to dopamine to do so. And if you do that, you can keep the therapeutic efficacy of levodopa. And everyone has thought that the only place in which you should express this enzyme, it's called aromatic acid decarboxylase (ADC), is the striatum, because this is where everyone has thought dopamine is doing its business to minimize the motor symptoms of the disease.
And certainly in animal models, if you boost ADC expression in the striatum, it works. The trouble is that in humans, the striatum has grown in parallel with the cerebral cortex. So it's a massive structure. It was extremely difficult to use a gene therapy that would effectively cover the full volume of the striatum in humans. And as a consequence, the clinical trial that was attempted to deliver ADC to the striatum had to be terminated because there were complications of trying to get so much virus out into the striatum. But the substantia nigra, where the cell bodies of dopaminergic neurons sit, is a very small structure. So what we did was we said, "Well, we think dopamine release either in the substantia nigra or the striatum should be effective in alleviating the symptoms of Parkinson's disease. And the last figure of the paper is an attempt to test that hypothesis.
So we use the same gene therapy strategy that had been attempted in humans, and we delivered ADC to the mesencephalon of mice that were fully Parkinsonian, and sure enough, delivery of ADC to the mesencephalon effectively boosted the symptomatic benefit of very low doses of levodopa and allowed mice to move again. So what this does is it points to a strategy that could be used in humans. It would be very easy with the gene therapy to cover the substantia nigra with a single surgical intervention, no indwelling wires, single procedure, and now your levodopa continues to work for however long the gene continues to express. Since the gene therapy for ADC already has been tested in humans, all we're essentially doing is talking about targeting it to a slightly different part of the brain. Safety's not so much of an issue. It's a part of the brain that's targeted by neurosurgeons all the time. As a matter of fact, it's a place that deep brain simulation comes very close to the substantial Niagara all the time. And so that may be the therapy for late-stage Parkinson's disease that could be very effective.
Amanda Dee: It's exciting that, already, it sounds like you have a step into the clinic from these findings.
D. James Surmeier: As a matter of fact, I've been emailing this morning with a neurosurgeon at Cornell.,it's a coauthor on the paper, [laughs] and we're talking about how to move this into the clinic. And we're looking for corporate partners now, and he's identified one he's, this is Michael Kaplitt. And Michael is a great neurosurgeon, and he's been very involved in gene therapy trials for Parkinson's disease in the past.
Amanda Dee: Speaking of collaboration and support, the Nature study was in part supported by the Michael J. Fox Foundation through which you also just received a $9 million grant from the Aligning Science Across Parkinson's initiative. How will this new funding aid your research?
D. James Surmeier: Yeah, this is a very generous award from the ASAP and the Michael J. Fox foundation. And we're all very excited about it. We put together a consortium of investigators from all around the world. I mean, really, I think the best and the brightest who are working on the basal ganglia, trying to understand how dysfunction in basal ganglia circuits leads to symptoms in much the same way that I've tried to describe today. Now, what happens when the striatum becomes dysfunctional? What happens when other parts of the basal ganglia become dysfunctional? And this team will allow us to pursue two key implications of the work. One is we really don't know very well how the motor symptoms arise. We know that loss of dopamine in the mesencephalon is a key trigger, but we don't know how that trigger leads to disruption of motor circuits in the brainstem.
And in the cortex, this team is going to allow us to sort that out. And that's important for a variety of reasons because it gives us other point pressure points, other places where we might be able to intervene in Parkinson's disease patients, to alleviate the motor dysfunction associated with the disease. The other really exciting thing about the consortium is that we're going to also pursue non-motor symptoms. So one of the key features of Parkinson's disease is that the disease is accompanied by disruption of sleep. And there are sort of two categories of sleep disruption. There's something called REM-based sleep disorder, where you act out your dreams. Normally when you sleep, your motor circuitry gets suppressed so that you can actively imagine running down the street, but you don't actually run down the street, you just imagine it, right?
In these patients that circuitry is not inhibited and they do all kinds of things that are often particularly harmful to their wives or husbands because it happens when they're asleep, but the more common sleep disruption is simply loss of slow wave sleep and excessive daytime sleepiness. And there's good reason to think not only does this impair the quality of life for Parkinson's disease patients, but it also may promote the disease progression. And why do I say that? There's good reason to think that during sleep, one of the things that happens is our brains clear misfolded proteins and sort of garbage. And so it's entirely possible that that sleep disruption is pushing the disease forward because it prevents the brain from cleaning itself, from healing itself while we sleep. And surprisingly, these mice that we've developed, which just have the disruption of mitochondrial complex I in dopaminergic neurons manifest a sleep disorder that is astonishingly like that that you see in most Parkinson's disease patients.
This is a little bit of a collaboration with somebody else that that was completely accidental. Fred Turek who's a circadian rhythms biologists here at Northwestern, his kids and my kids go to the same school. And we got to talking about this model. And I said, "You know, Fred, these mice seem to die very prematurely." And he said, "Jim, you know, if I sleep deprive a mouse for a couple of weeks, it has a high probability of dropping dead." And I said, "Fred, why don't you take some of these mice and go look to see whether they have a sleep disorder." And sure enough, about, I don't know, a month or so after he got the mice, I got this call and he said, "These mice have got as close to a Parkinsonian sleep disorder as I've ever seen." It led to not just a collaboration with Yang Dan at UC Berkeley, who's going to be studying sleep disorder, but Fred and I got an award from the Department of Defense to study the circuit mechanisms underlying the sleep disorder in these mice as well. But it was all based upon Fred's expertise and Fred's insight into not just the sleep disorder of PD but what ultimately might cause it and what are the consequences for the progression of the disease. This is what leads to breakthroughs is getting together and working as a team and communicating effectively.
Amanda Dee: Well, thank you so much for sharing these breakthroughs on Parkinson's research. I am sure that we will be talking to you again soon, there's so much to look forward to. And thank you for your time.
D. James Surmeier: It was my pleasure. Thanks so much for having me.
Amanda Dee: Thank you for listening to this episode of Breakthroughs. Find more episodes and earn Continuing Medical Education credit by visiting feinberg.northwestern.edu and searching "Breakthroughs."
Continuing Medical Education Credit
Physicians who listen to this podcast may claim continuing medical education credit after listening to an episode of this program.
Target Audience
Academic/Research, Multiple specialties
Learning Objectives
At the conclusion of this activity, participants will be able to:
- Identify the research interests and initiatives of Feinberg faculty.
- Discuss new updates in clinical and translational research.
Accreditation Statement
The Northwestern University Feinberg School of Medicine is accredited by the Accreditation Council for Continuing Medical Education (ACCME) to provide continuing medical education for physicians.
Credit Designation Statement
The Northwestern University Feinberg School of Medicine designates this Enduring Material for a maximum of 0.5 AMA PRA Category 1 Credit(s)™. Physicians should claim only the credit commensurate with the extent of their participation in the activity.
Disclosure Statement
D. James Surmeier, PhD, disclosed financial relationships with Lundbeck and Neurodon (consulting), and Cavalon Therapeutics (ownership). Course director, Robert Rosa, MD, has nothing to disclose. Planning committee member, Erin Spain, has nothing to disclose. Feinberg School of Medicine's CME Leadership and Staff have nothing to disclose: Clara J. Schroedl, MD, Medical Director of CME, Sheryl Corey, Manager of CME, Allison McCollum, Senior Program Coordinator, Katie Daley, Senior Program Coordinator, and Rhea Alexis Banks, Administrative Assistant 2.
Credit opportunity coming soon.